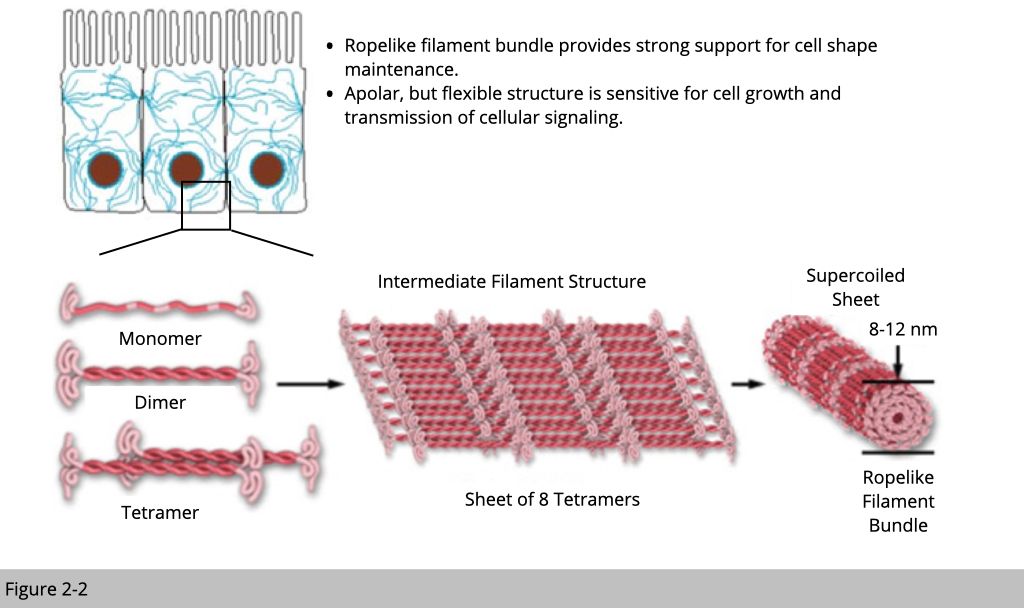
In the lecture one we have seen the immunostaining of intermediate filament. The distribution of intermediate filament forms a network within the cell. It was often served an anchor node to tight things together. Intermediate filaments are built from monomers that associate with each other to form dimers. Pairs of dimers then associate in an anti-parallel fashion to form staggered tetramers. Moreover, intermediate filaments are apolar, so intermediate filaments are much “stable” compared to actin or tubulin. Intermediate filaments are the toughest and most durable material for building up cells of the three types of cytoskeletal filaments.
The subtypes of intermediate filament.
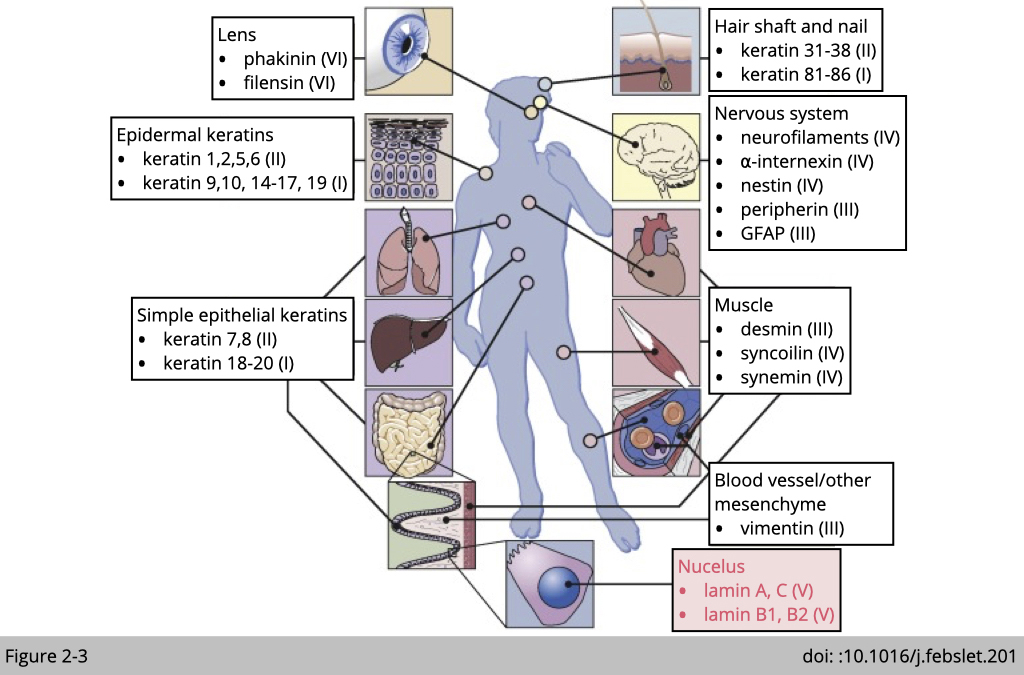
There are six major types of intermediate filament could be found in mammalian cells. Most cells have their own unique intermediate filament. Such as neurofilaments in neuron cells we have talked about on the lecture one. Within intermediate filament family, type V of intermediate filament, lamin A, B, and C are widely expressing in most of the mammalian cells. They are critically important for the structural properties of the nucleus.
Lamins locate in the nuclear.
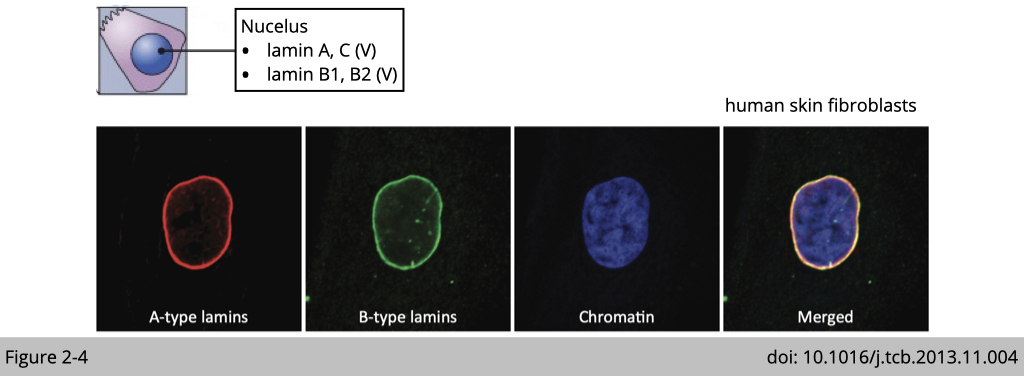
Nuclear lamins interact with inner nuclear membrane proteins to form the interior of the nuclear envelope. From the immunofluorescence (IF) staining on human skin fibroblasts, you can see the majority of lamin A/C and B are located in nuclear envelope. Yet, there are also some spot-like staining of lamin A/C within the nucleoplasm, where they are associated with heterochromatin and responsible for transcription regulation.
Question: Based on the distribution and structure of lamins (type V intermediate filament) in the nucleus, what do lamins function there?
Clue #1: Nuclear abnormalities in cells from old individuals associating with lamin A/C deficiency.
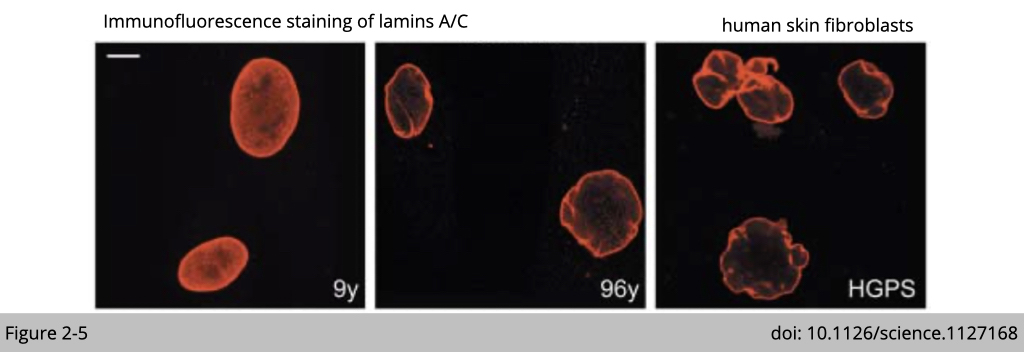
The decrease expression of lamin A/C has been reported in skin fibroblasts isolated from the elderly and the patients suffering from the premature aging such as Hutchinson-Gilford progeria syndrome (HGPS). The decreased expression of lamin A/C is also associated with the destructive morphology of nuclear envelope as you can see in the Figure 2-5.
Moreover, the knockdown of lamin A/C reassemble the nuclear abnormalities in culturing cell and phenocopy the aging phenotype in various mouse models.
Clue #2: Lamin pathology was reported in human Alzheimer’s disease.
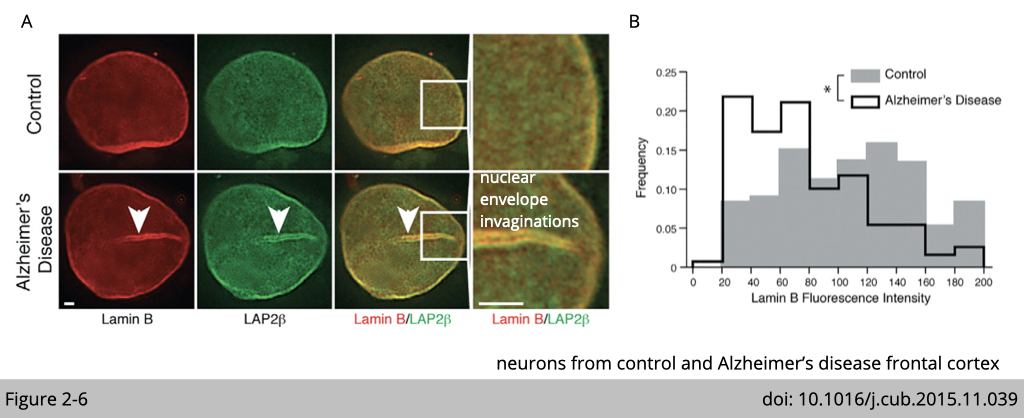
The reduction of lamin is also observed in neuron cells isolated from Alzheimer’s diseases patients. Similar to aging cell, the abnormal nuclear envelope invaginations was observed in neurons from control Alzheimer’s disease frontal cortex.
Clue #3: The pathologies of cardiac and skeletal muscle are dominant in laminopathies.
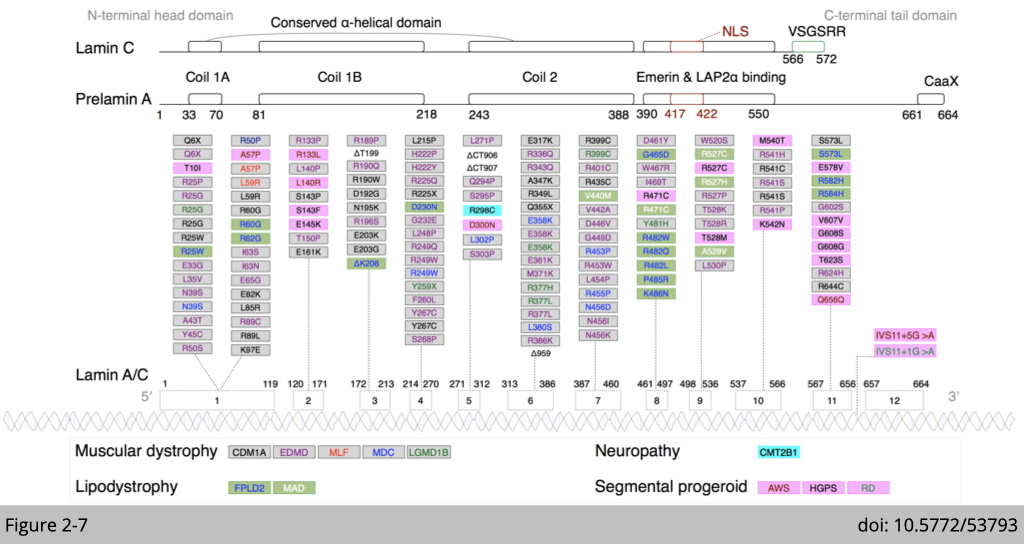
Various mutation of Lamin A/C have been identified in human. Those mutations are all associated with certain “atrophy” phenotype.
Clue #4: Laminopathies: Hutchinson–Gilford progeria.
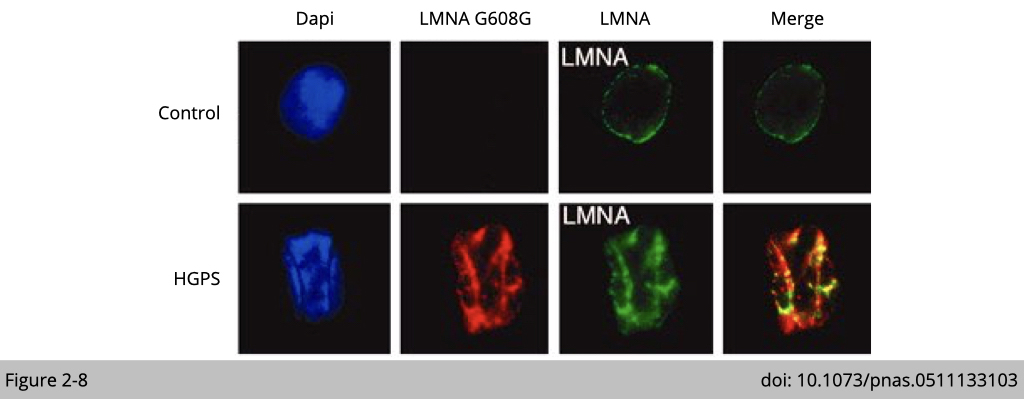
The fibroblast expressing the mutation of Lamin A/C identified in progeria patients show severe nuclear envelope deformations and invaginations (Figure 2-8). Moreover, over-expression of lamin mutation reassembles the nuclear abnormalities in culturing cell.
Based on those observations, we could conclude that one of the lamins function is to maintain the stability of nuclear shape, which might be responsible to protect material stored in nucleus such as DNA. We will discuss this more in our journal club later.
A quick summary of intermediate filaments, because Intermediate filaments are the toughest and most durable material within cytoskeleton family. It is a good material for building up cell and provide force for cell division.

Unlike the stability of intermediate filament, microtubules and actin microfilaments are constantly polymerized and depolymerized. Moreover, they have their own molecular motors associated with them. They are also responsible for cargo transportation within cell. The polymerization and depolymerization of actin filaments and microtubules generate dynamic network and, together with their molecular motors, guide the organization of cellular components.
Tubulin 101, microtubules are composed of α- and β-tubulin. Microtubules have a polarity in which the (-) end is far less active than the (+) end. Both the α-tubulin and β-tubulin must bind to GTP for polymerization, but once bound, the GTP bound to α-tubulin does not move. On the other hand, GTP bound in the β-tubulin may be hydrolyzed to GDP. Once exposure of terminal GDP-bound subunits of microtubules will lead to disassemble.
The tubulin superfamily.
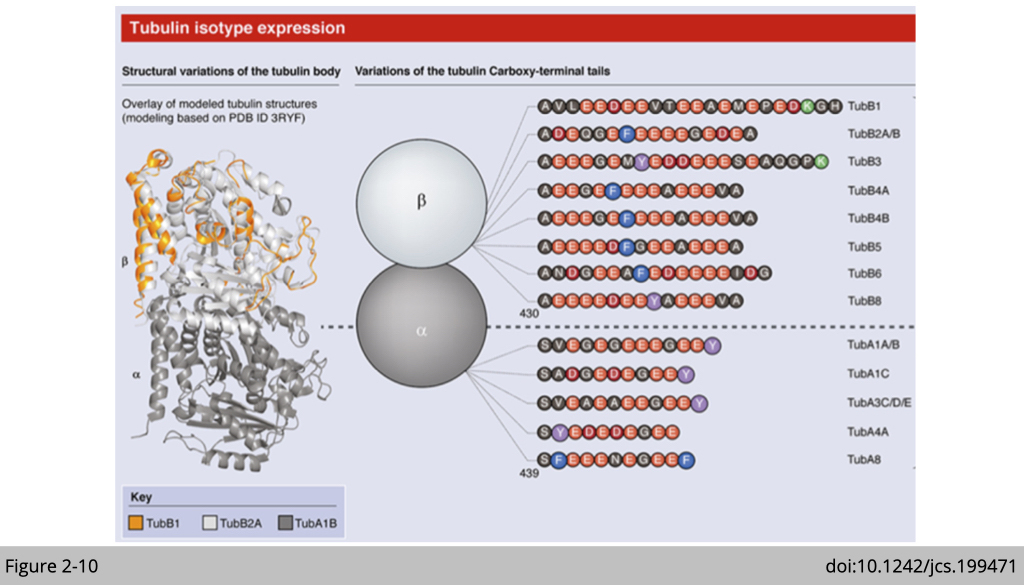
In eukaryotes there are six members of the tubulin superfamily including (alpha-(α), beta-(β), gamma-(γ), delta-(δ), epsilon-(ε), and zeta-(ζ) tubulins), although not all are present in all species. So far, seven α-tubulin and nine β-tubulin isotypes have been reported in humans. These tubulin isotypes share a high degree of sequence similarity. The variation in the sequences are concentrated at the unstructured C-terminal tail, especially in the last 15–20 amino acids. The c-terminal tails of tubulin are exposed outside of microtubule and are the major site of tubulin post-translational modification which regulates tubulin's binding interactions with other proteins.
Post translational modifications of tubulin.
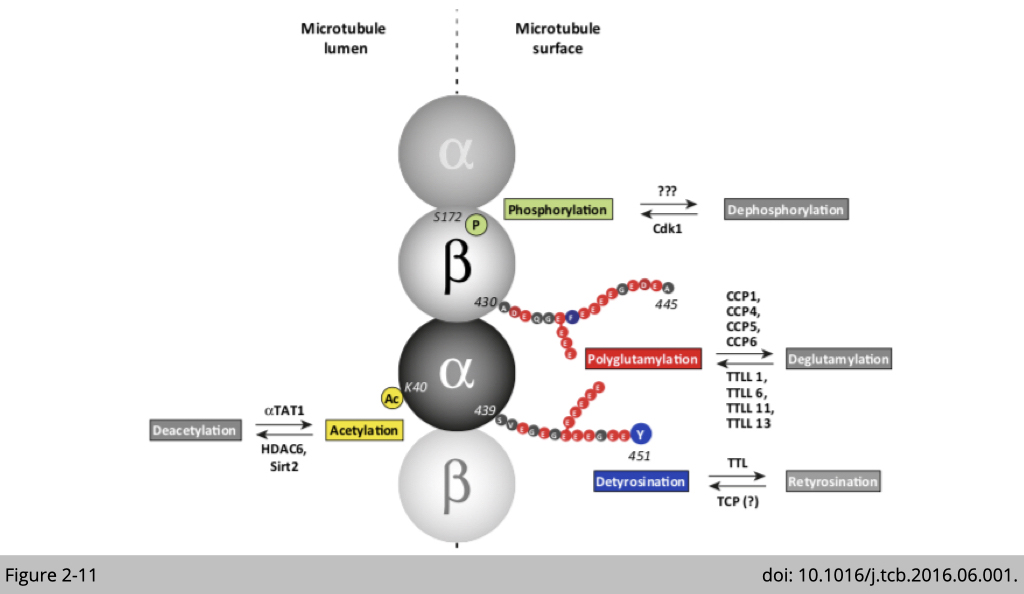
The post translational modifications (PTMs) of tubulin include phosphorylation, glutamylation, detyrosination, and acetylation listed in Figure 2-11. Methlyation, acetylation, phosphorylation, amination, palmitoylation, ubiquitination, glycosylation, arginylation, sumoylation, and succination are also been reported in tubulin. Specific patterns of PTMs can program the microtubules to perform specialized functions, either by modulating the mechanical properties of microtubules or by influencing the interaction of microtubules with its associated protein such as motor protein for cargo transportation.
Traffic control inside the cell: post-translational modifications of tubulins.
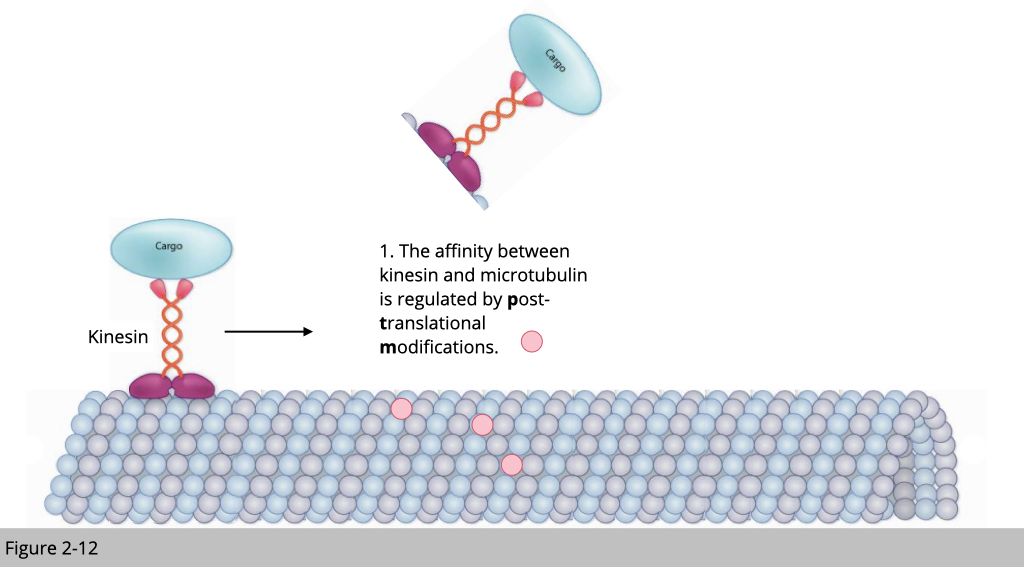
Note that microtubulin plays an important role for cargo transportation within cell. How does our cell regulate the traffic? In the real world, we know that car move faster in the paved road than the dirt road. By using different type material to buildup a road, we can control the speed of motor. Second, the traffic control in the city was often coordinated by different traffic signs or electromechanical controllers. For example, the green light is a universal sign for moving and the red light for stopping.
Similarly, our cell can control the cargo transportation by modifying the tubulin. For example, excessive polyglutamylation could damage the microtubulin track and then stop the tubulin motor protein- kinesin transportation.
Microtubule-associated proteins.
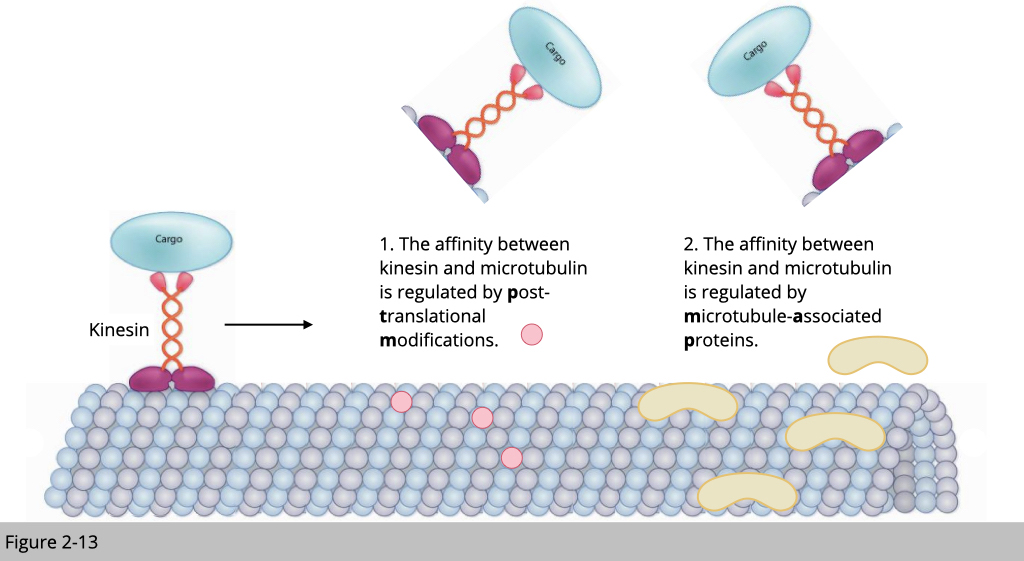
Microtubule is also known to interact with a lot of proteins. Those proteins can either inhibit or recruit motor proteins, such as kinesin loading on the microtubule track.
Tauopathy.
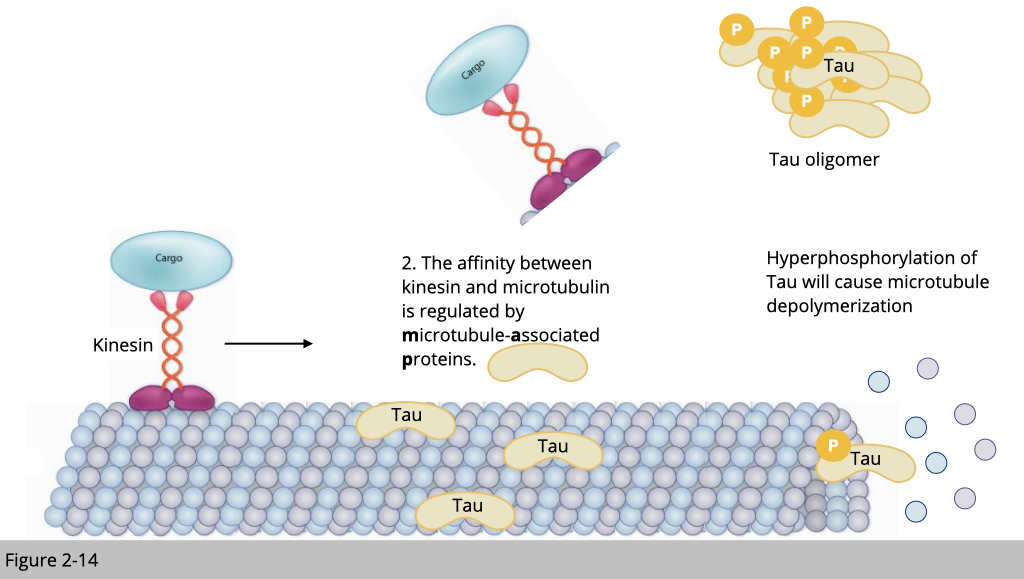
For example, TAU is one of the most studied microtubule-associated proteins and highly express in neuron. The hyperphosphorylated TAU identified in Alzheimer’ disease patients is known to cause the microtubule depolymerization, which might contribute to pathologies of Alzheimer’ disease.
In summary, the dynamic feature of microtubules provide the cell ability to quickly reconstruct its shape, the speedy network for transportation and also the force for cell movement.
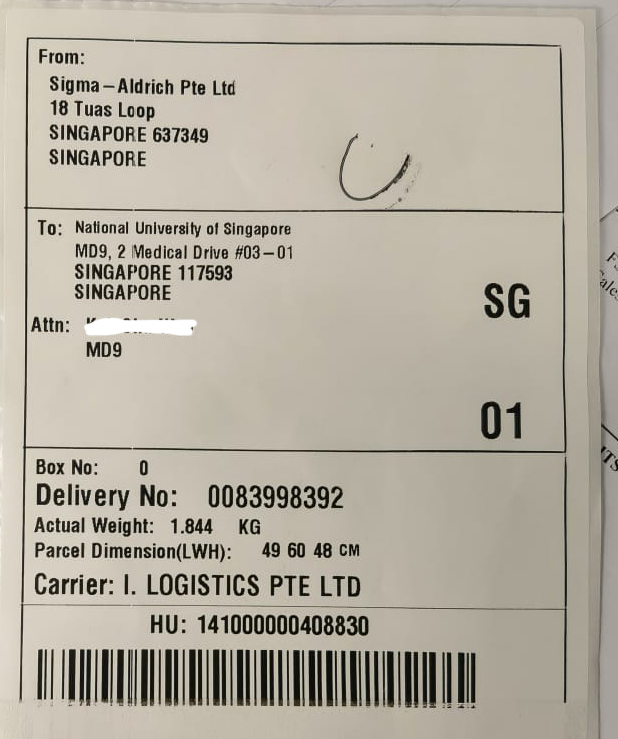
In the modern world, logistics companies use the barcode to process a shipment. The shipping barcode is scanned at each phase of the delivery until it reaches customers. We can easily track and locate shipments via barcode. Yet, we are not the first ones to invent this system.
Our cells have used a similar barcode system for transportation for a long time, such as nuclear localization signals (NLS) peptides and secretory signal peptides (SPs) are well-known sequence motifs targeting proteins for relocation into nuclei and translocation across the endoplasmic reticulum membrane respectively. Surprisingly, a similar mechanism has also been found in the translocation of RNA. We will review how the unique barcode in β-actin RNA authorizes its supreme power over other members next.
Structural characteristics of F-actin and actin-associated proteins.
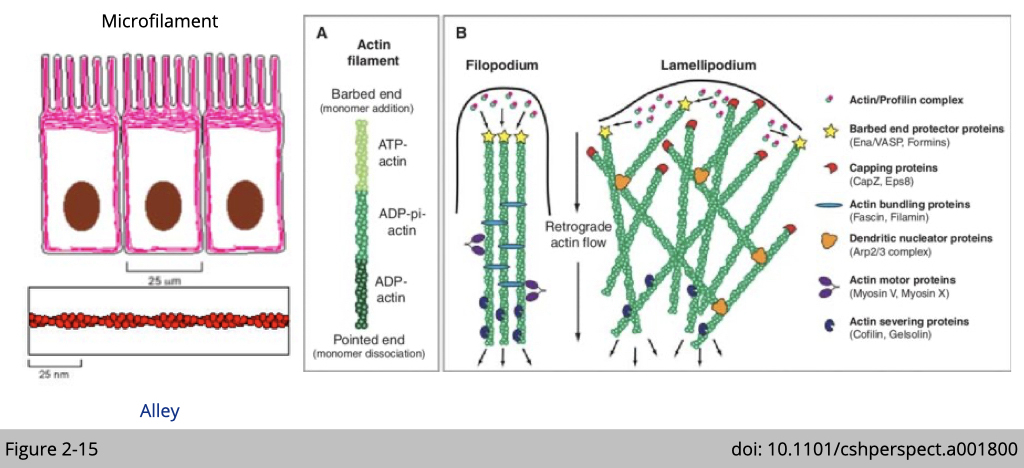
In a migrating cell, actin filaments polymerize at the leading edge, and flow back into the body of the cell (retrograde flow). Filaments grow at barbed end, whereas depolymerization occurs at the pointed end. Noted that there are many actin-associated proteins which regulate the architecture of actin filament in dynamics. For example, actin-related proteins-2/3 (ARP2/3) complex (orange object in Figure 2-15) is one of three known actin nucleators in eukaryote and is responsible for the actin nucleation and branching.
Network architecture and mechanics of actin filament.
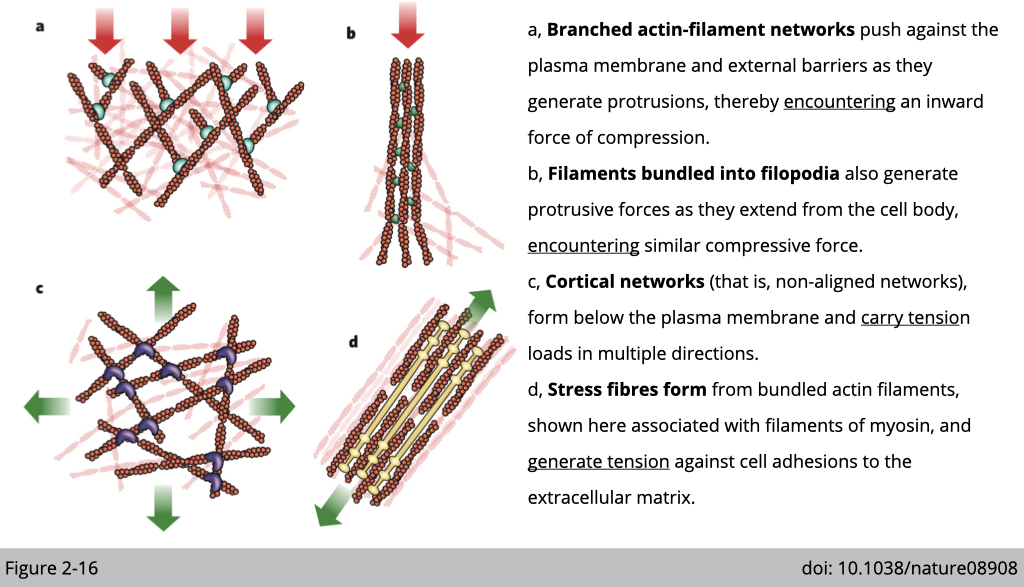
Actin filament can produce pushing (protrusive) forces through coordinated polymerization of multiple actin filaments or pulling (contractile) forces through sliding actin filaments along bipolar filaments of myosin II. Actin filament is also the tension sensor in the cells. The tension generated from external or internal force will affect different protein binding to actin and then change its structure.
In general, actomyosin (the protein complex is composed of actin filament and its motor protein, myosin) is the major force generator in cell.
Actin has very high selective pressure for sequence conservation.
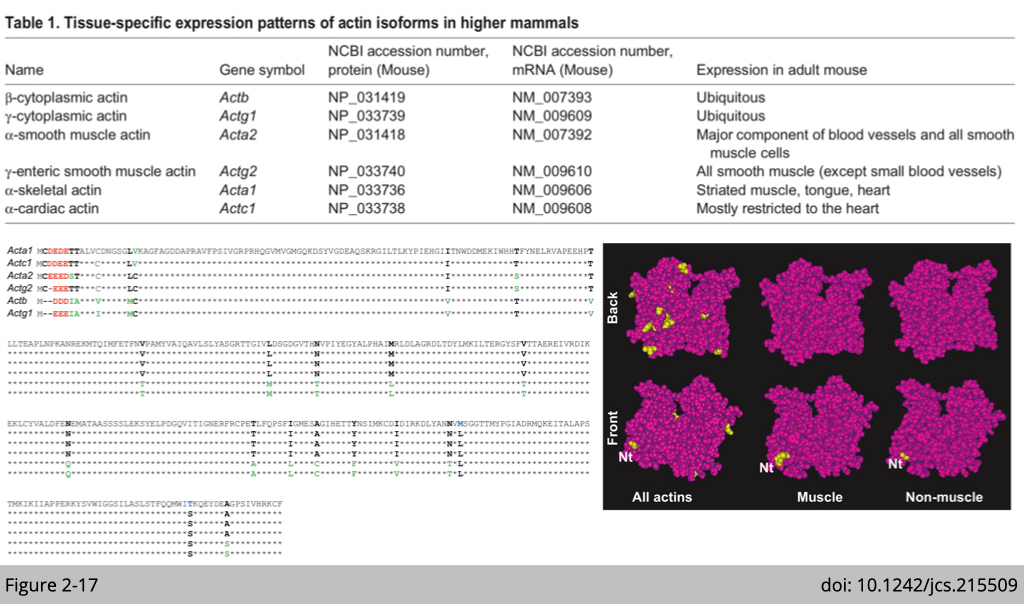
Unlike intermediate filament and tubulin, actin does not have many isoforms. All actin isoforms differ markedly within the three or four residues at their extreme N-terminus; a region of the actin molecule that is exposed on the surface of actin monomers and filaments. Notably, however, these substitutions are largely conservative, and result in only a small overall change in the negative charge in this region.
Question: What makes ß-actin so unique?
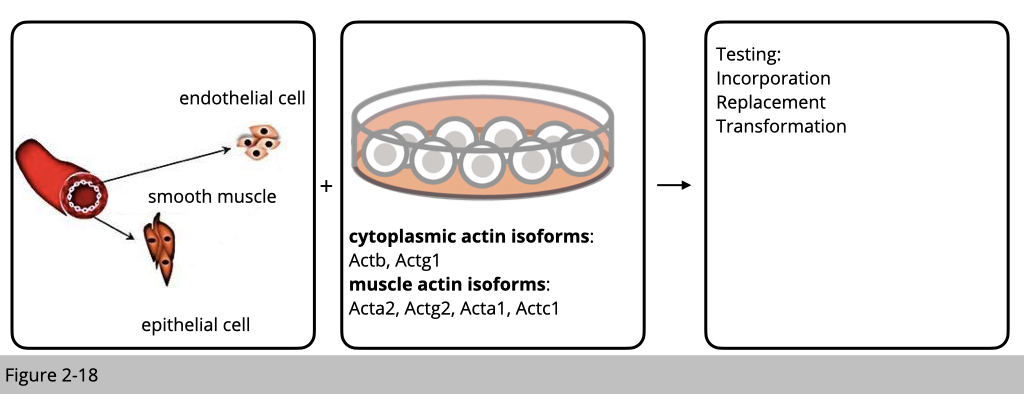
Actin is one of the most highly conserved proteins and plays crucial roles in many vital cellular functions. It has been previously suggested that the existence of such similar actin genes is a fail-safe mechanism to preserve the essential function of actin through redundancy. But is it really simple like that?
It was observed relatively early that muscle actin isoforms cannot replace the cytoplasmic actins in certain cellular structures and conversely, cytoplasmic actins cannot replace the muscle actins. The experiment is designed as such that cells from different linages were transfected with each actin isoform to see whether the exogenous over-expressing actin isoform can incorporate into the existed actin filament or transform cell to the other cell type. Or using RNAi knockdown of different isoforms, to see whether over-expression of the other isoform can rescue the phenotype in cell movement. Therefore, those in vitro experiments suggest that actin isoforms play divergent roles in cell.
Non-redundant biological roles of mouse actin isoforms.
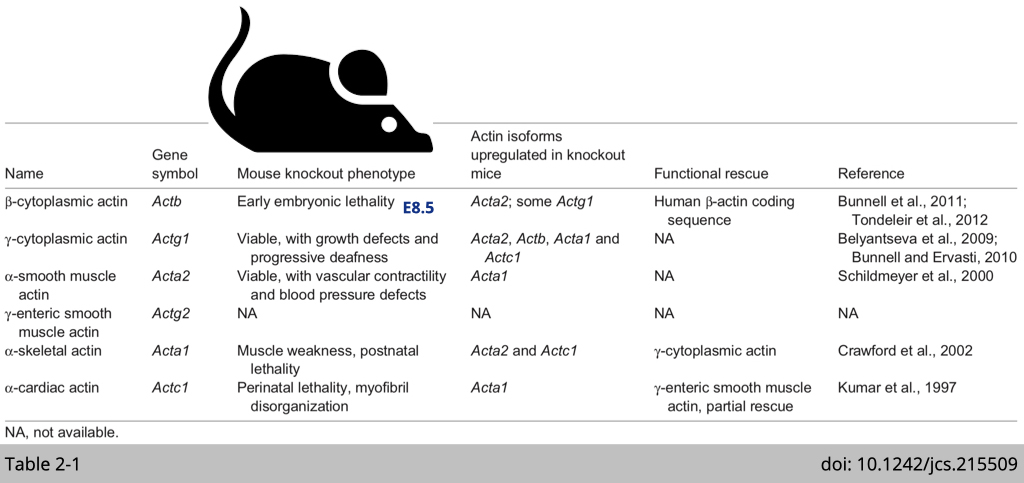
In all cases, knockout of a single actin isoforms leads to the upregulation of the other isoforms to maintain a constant amount of actin protein, suggesting there is a ‘dosage-sensing’ mechanisms that ensure the constitutive abundance of the total actin. This dosage, however, does not result in total functional compensation in most cases. Because each knockout mouse still has their unique phenotype, over-expression of the other isoform of actin only partially rescue the phenotype.
For example, mice lacking α-cardiac actin are embryonic lethal and show disorganized myofibril organization in the heart tissue. Heart-specific expression of γ-smooth muscle actin in these mice, rescues the perinatal lethality and enables these mice to survive to adulthood. However, the hearts in these transgenic mice still show hypertrophy. These experiments suggest that while γ-smooth muscle actin can compensate for the loss of α-cardiac muscle actin, there is non-redundant biological function of α-cardiac muscle actin can not be replaced by γ-smooth muscle actin.
Within all the knockout mice have been generated, disruption of the β-actin gene in mice causes by far the most severe phenotype, leading to early embryonic lethality. What makes ß-actin so unique?
β-actin gene structure.

As we know that the protein of all actin isoforms are very similar. The difference is only within the three or four amino acid at their extreme N-terminus. Yet, these six actins are encoded from six unique chromosome loci. Their nucleic acid sequences are very different from each other, which could drive different level of expression pattern.
Gene expression was regulated into three different levels.
- The first level is at the regulation of transcription which different promoters recruit unique transcription factor complex to drive differential gene expression.
- The second level is at the regulation of mRNA stability and translation. The intron of a gene which will affect the mRNA splicing efficiency. The untranslated region on 5’ and 3’ end of mRNA could be the target for microRNA which will then affect mRNA stability and are also important for ribosome loading which affect translation efficiency.
- The third level is at the regulation of protein stability.
The nucleotide sequence of β-actin, rather than its amino acid sequence, determines its essential role in vivo.
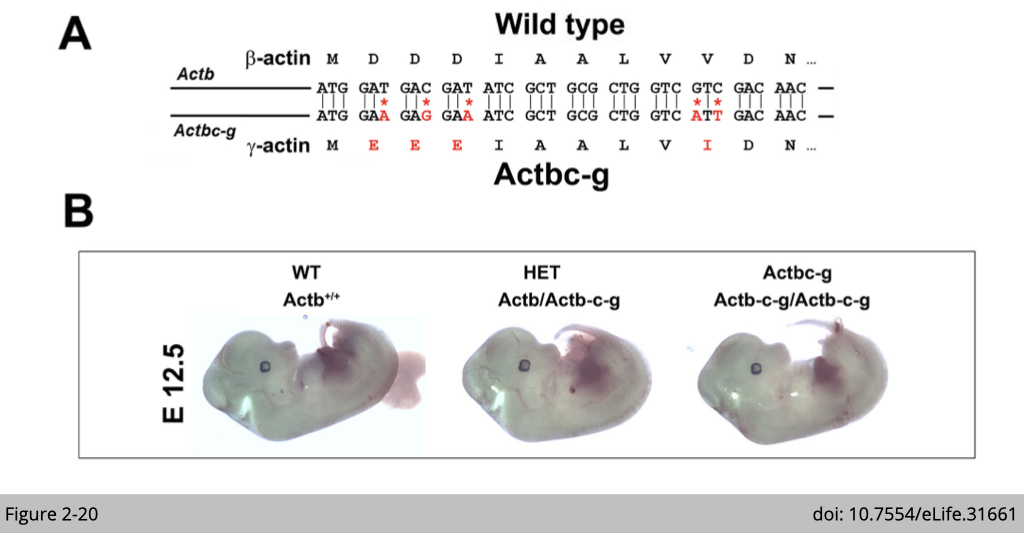
One of the pioneer experiment, Anna Kashina’s group at University of Pennsylvania did was to generate a mouse lacking β-actin protein by editing β-actin gene to encode γ-actin. We have talked about this that β- and γ- actin are nearly identical proteins except for four residues within their N-termini. Yet, their coding sequences differ much more significantly, by nearly 13%, due to silent substitutions of amino acid from synonymous nucleotide. Moreover, their non-coding sequence are almost different.
So what Anna did is that they used CRISPR/Cas9-mediated gene editing to introduce five point mutations into the native mouse β-actin gene (Actb), altering it to encode γ-actin protein without changing any of the features of the rest of the gene sequence. The expression of β-actin protein now are replaced by γ-actin protein.
Note that β-actin knockout mice are embryonic lethal and transgenic over-expression of γ-actin could not rescue the lethality. Strikingly, this γ-actin knockin β-actin mice appeared completely healthy, viable, and fertile with no signs of deficiencies previously reported in any of the β-actin knockout mouse models.
This piece of genetic study provide the solid evidence that β-actin nucleotide sequence, rather than amino acid sequence, defines its essential role in vivo.
Differences between β-actin and γ-actin at different levels.
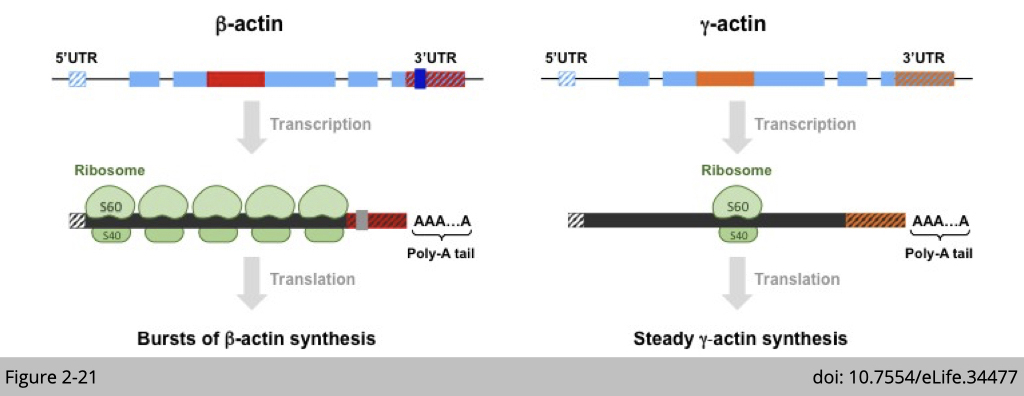
So, how can differences in the nucleotide coding sequences through silent substitutions account for the divergence of actin functions? One of the major known effects of the coding sequence relates to the potential differences in codon usage and secondary structure of mRNA, which can lead to different ribosome translocation dynamics, and thus to different rates of translation. In the case of β- and γ-actin, coding sequence differences give rise to a different secondary structure. A stable loop is present in the 5′ coding region of γ-actin, but is not prominent in the corresponding region of β-actin, leading to slower ribosome translocation over γ-actin mRNA in this region.
Moreover, at the level of genes, two elements are conserved features that are found in both birds and mammals: intron 3 (shown in red for β-actin and orange for γ-actin) and the 3´ untranslated region (3´ UTR) are different. The intron 3 of γ-actin has been reported to cause non-sense splicing which indirectly cause mRNA instability and interfere with translation machine loading.
Interestingly, The 3’UTR of β-actin has been intensively studied and it is a perfect example demonstrating on how a non-coding region affect a gene’s function. The first observation is that, even though β- and γ-actin are both ubiquitously expressed in cytoplasm and coexist at comparable levels in many cell types, a substantial fraction of β-actin mRNA (an estimated 10%) is localized to the leading edge in motile cells. Later studies found that β-actin has a sequence (labelled in grey in Figure3-8) that directs the mRNA molecules to the periphery of the cell.
The nucleotide sequence of β-actin, rather than its amino acid sequence, determines its essential role in vivo.
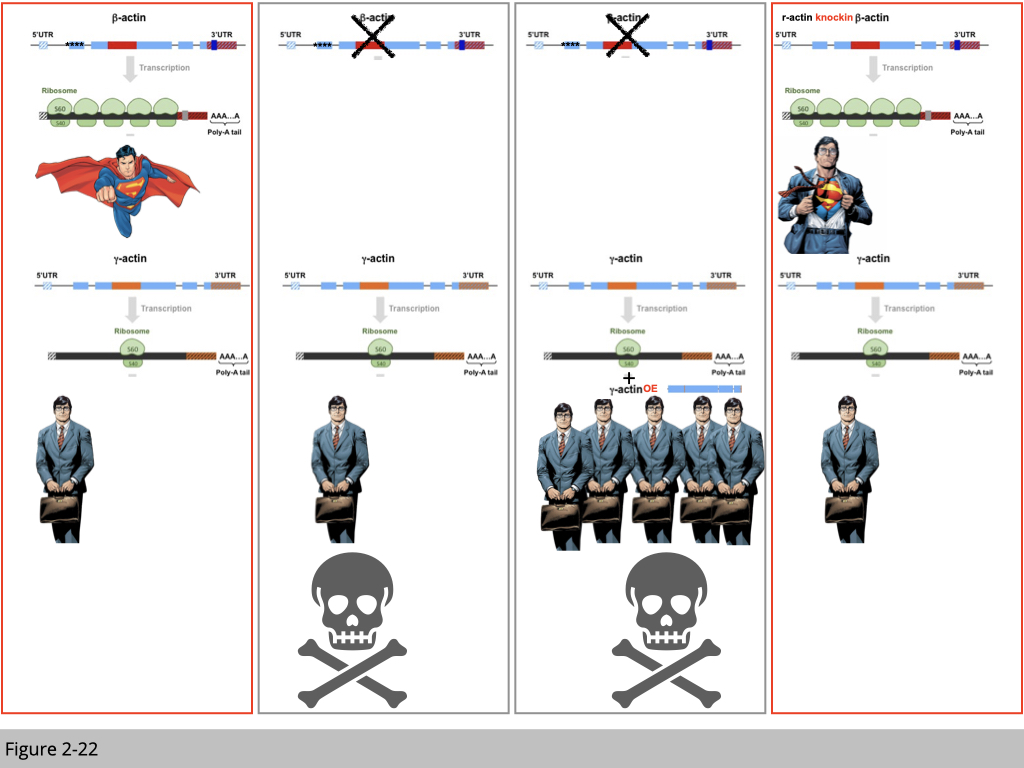
Based on the genetic study, firstly we know that β-actin is superior than other members in the family of actin. The knockout of β-actin leads to early development defect.
Second, each actin is located on the different chromosome loci and has distinct RNA structure. Yet, they are highly conserved in protein sequence.
Third, the mechanism responsible for the different mRNA structures is the translation efficiency. Even though the mRNA level is similar between β- and γ-actin, there is more β-actin protein in the cell compared to γ-actin. Yet, over-expression of γ-actin could not rescue the embryonic lethality due to β-actin deficient. From this over-expression experiment, we can conclude that the superior of β-actin is not due to its abundance. Do you agree?
The last piece of knockin experiment is done by Anna’s group that they transgenically swap four amino acids from β-actin for γ-actin in mice. Now this β-actin mRNA will be translated into γ-actin protein, yet will maintain all the features of β-actin mRNA. This knockin mouse is alive without any β-actin protein expressed in its body.
What’s this tell us? There is something unique of β-actin mRNA making its so special and essential. The simple analog is that not all reporters will be the superman. Only Clark Kent has super-power to be the superman. So what’s special of β-actin mRNA structure? Why the Clark Kent can be transformed to a superman after he wears his superman cape?
Transgenic insertion of the lacZ reporter into the β-actin 3′UTR causes pre-implantation lethality.
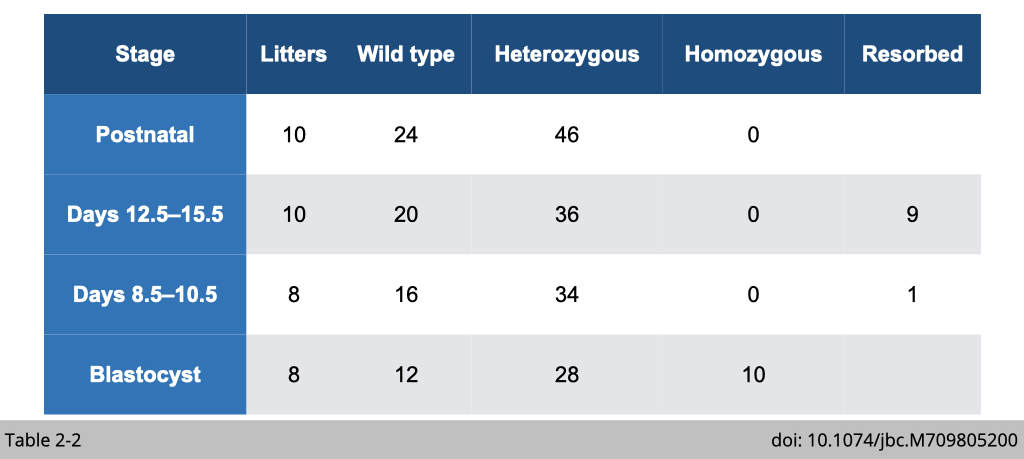
Based on the β-actin mRNA structure, there are couple regions is “evolutionary conserved” from bird to mammal of β- and γ-actin. Those are 5’UTR, intron 1 and 3’UTR. I have told you that, 5’UTR is important for ribosome loading, intron 1 is important for mRNA splicing which responsible for mRNA stability of γ-actin, and 3’UTR is required for β-actin mRNA location. Yet, we know that the unique of β-actin is not due to its abundance.
So why does it matter for a RNA to be at the right place? In the other words, how does the 3’UTR affect β-actin’s location and in turns regulate its function?
- Observation 1: the transcription of β-actin from the β-actin 3’UTR disrupted cells shows a 17% reduction of its expression. Later, John Clark's group found that disruption of 3’UTR affect the methylation on CpG island of β-actin promoter.
- Observation 2: the disruption of β-actin 3’UTR phenocopied embryonic lethality reported in β-actin knockout mice. (Table 2-2)
From the biochemistry and genetic studies, we can conclude that 3’UTR is one of the most important region to make β-actin as β-actin. Actually, I think it will be very cool if someone can engineer the 3’UTR of γ-actin and to see whether the modified γ-actin can rescue the lethality in β-actin knockout mice. Then the conclusion will be solid as a diamond.
Localization of β-Actin mRNA directs cell migration.
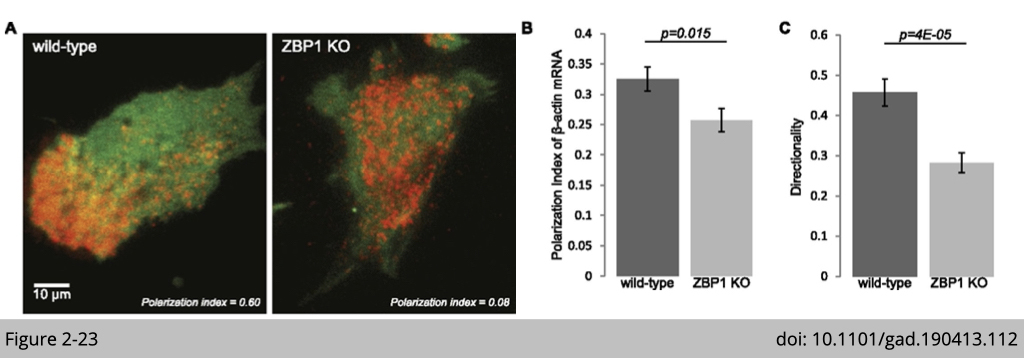
The polarity distribution of β-actin is dependent on zipcode-binding protein 1 (ZBP1). The zipcode sequence on β-actin 3’UTR bind to ZBP1. ZBP1 binds and transports β-actin mRNA to the focal adhesion compartment. As shown in Figure 3-11, β-actin is labeled with red fluorescence. In the wildtype cells induced for migration, β-actin was located on the leading edge of motile cell. Yet, in the ZBP1 knockout cell, β-actin totally lose its polarity distribution.
There are different ways you can trace mRNA distribution in a cell. The most common way is in situ hybridization (RNAscope Technology is a novel in situ hybridization (ISH) assay. https://acdbio.com/science/how-it-works). Yet, this paper used a chemical to genetically label β-actin mRNA in vivo. The MS2 stem loops was fused with the 3’UTR of β-actin. Then the MS2 stem loops will be recognized by MS2 coated protein which fused to red fluorescence protein. This detection is specific and the signal could be amplified (The green signal here is from another transgene, GFP to label cytoplasm).
What we have learned from the regulation of β-actin 3’UTR could impact more in our daily life. For example, nowadays when we talked about RNA therapies that a fragment of sense or antisense of gene or miRNA is synthesized in vitro and then introduce it to cell or body. If we could identify more of this type of silent code in RNA, we could engineer more effective delivery systems for RNA-based therapies.
Final piece of matching game of cytoskeleton. Actin filament is not only served as cellular blocks, but also plays an important role for internal force generation and external force sensor. We will discuss about its role for cargo tracking in the next lecture.